What I Can Learn From Atomic Structures is a vast field that offers insights into the very foundation of matter and its interactions, providing a gateway to understanding everything from material properties to biological processes. At LEARNS.EDU.VN, we delve into the fascinating world of atomic structures and their implications, offering a wealth of knowledge and resources to help you unravel the mysteries of the universe. Explore how understanding atomic structures enhances skills in scientific analysis, problem-solving, and innovation, ultimately fostering a deeper appreciation for the interconnectedness of science and the world around us through our chemistry courses.
1. Understanding the Basics of Atomic Structures
Atomic structure, at its core, is the arrangement of protons, neutrons, and electrons within an atom. This arrangement dictates how an atom interacts with other atoms to form molecules and materials. Here’s a breakdown of key concepts:
- Protons: Positively charged particles located in the nucleus, determining the element’s atomic number.
- Neutrons: Neutral particles also in the nucleus, contributing to the atom’s mass.
- Electrons: Negatively charged particles orbiting the nucleus in specific energy levels or shells.
Understanding these components and their arrangement is fundamental to grasping chemical properties and behaviors. As stated by Linus Pauling, a pioneer in the field of chemical bonding, “The nature of the chemical bond is the problem at the heart of all chemistry.” This highlights the critical importance of understanding how atoms interact.
1.1. Electron Configuration and Energy Levels
Electrons don’t just orbit the nucleus randomly; they occupy specific energy levels or shells. Each shell can hold a certain number of electrons:
- First shell: Up to 2 electrons
- Second shell: Up to 8 electrons
- Third shell: Up to 18 electrons (though often follows the octet rule)
The electron configuration describes how electrons are distributed among these energy levels and sublevels (orbitals). This configuration dictates the chemical behavior of an atom. For example, atoms with a full outer shell (like noble gases) are generally inert, while those with incomplete outer shells are more reactive.
1.2. The Periodic Table: A Map of Atomic Structures
The periodic table is an organized chart of all known elements, arranged by their atomic number and electron configuration. Elements in the same group (vertical column) have similar chemical properties because they have the same number of valence electrons (electrons in the outermost shell).
For instance, Group 1 elements (alkali metals) all have one valence electron, making them highly reactive. Group 17 elements (halogens) have seven valence electrons, making them eager to gain one more to achieve a full outer shell, also making them very reactive.
Alt Text: A simplified diagram of the periodic table illustrating the arrangement of elements based on their electron configurations.
2. Why Study Atomic Structures? Practical Applications
The knowledge of atomic structures isn’t just theoretical; it has profound practical applications across various fields.
2.1. Materials Science: Designing New Materials
Understanding atomic structures allows scientists to design new materials with specific properties. For example:
- Strength: The arrangement of atoms in a crystal lattice determines the strength of a material. Materials with tightly packed, ordered structures are generally stronger.
- Conductivity: The ability of electrons to move through a material determines its conductivity. Metals have loosely held electrons that can move freely, making them good conductors.
- Semiconductors: Materials like silicon have a unique atomic structure that allows their conductivity to be controlled, making them essential for electronics.
By manipulating atomic structures, scientists can create materials with tailored properties for specific applications, from stronger alloys for aerospace to more efficient semiconductors for computers.
2.2. Chemistry: Understanding Chemical Reactions
Chemical reactions involve the rearrangement of atoms and molecules. Understanding atomic structures allows chemists to predict how reactions will occur and design new reactions.
- Bonding: Atoms form bonds by sharing or transferring electrons to achieve a stable electron configuration. Knowing the electron configuration of atoms allows chemists to predict what types of bonds they will form.
- Reactivity: The reactivity of a substance depends on the ease with which its atoms can form or break bonds. Understanding atomic structures helps predict reactivity.
- Catalysis: Catalysts speed up chemical reactions by providing an alternative reaction pathway. Understanding how catalysts interact with molecules at the atomic level is crucial for designing effective catalysts.
2.3. Biology: Understanding Biological Molecules
Biological molecules like proteins, DNA, and carbohydrates are complex structures made of atoms. Understanding their atomic structures is crucial for understanding their function.
- Protein Folding: The sequence of amino acids in a protein determines its three-dimensional structure, which is essential for its function. Atomic-level details of protein structure reveal active sites and binding pockets.
- DNA Structure: The double helix structure of DNA, with its specific base pairing (adenine with thymine, guanine with cytosine), is essential for its role in storing and transmitting genetic information.
- Enzyme Function: Enzymes are biological catalysts that speed up biochemical reactions. Understanding the atomic structure of enzymes reveals how they bind to substrates and catalyze reactions.
2.4. Medicine: Designing New Drugs
Understanding atomic structures is crucial for designing new drugs that target specific molecules in the body.
- Drug Binding: Drugs work by binding to specific target molecules, like proteins or enzymes. Understanding the atomic structure of the target molecule allows scientists to design drugs that bind with high affinity and specificity.
- Drug Metabolism: The body metabolizes drugs, breaking them down into inactive or less active forms. Understanding the atomic structure of drugs allows scientists to predict how they will be metabolized and design drugs that are more resistant to metabolism.
- Personalized Medicine: Understanding the genetic variations between individuals allows for personalized medicine, where drugs are tailored to an individual’s specific genetic makeup. This requires a deep understanding of atomic structures and molecular interactions.
3. Methods for Determining Atomic Structures
Several powerful methods are used to determine the arrangement of atoms in molecules and materials. These methods provide scientists with detailed information about atomic structures.
3.1. X-ray Crystallography
X-ray crystallography is a technique used to determine the atomic and molecular structure of a crystal. In X-ray crystallography, a beam of X-rays strikes a crystal, causing the X-rays to diffract into many specific directions. By measuring the angles and intensities of these diffracted beams, a three-dimensional picture of the density of electrons within the crystal can be produced. From this electron density, the positions of the atoms in the crystal can be determined.
Most of the structures included in the Protein Data Bank (PDB) archive were determined using X-ray crystallography. For this method, the protein is purified and crystallized, then subjected to an intense beam of X-rays. The proteins in the crystal diffract the X-ray beam into one or another characteristic pattern of spots, which are then analyzed (with some tricky methods to determine the phase of the X-ray wave in each spot) to determine the distribution of electrons in the protein. The resulting map of the electron density is then interpreted to determine the location of each atom. The PDB archive contains two types of data for crystal structures. The coordinate files include atomic positions for the final model of the structure, and the data files include the structure factors (the intensity and phase of the X-ray spots in the diffraction pattern) from the structure determination. You can create an image of the electron density map using tools like the Astex viewer, which is available through a link on the Structure Summary page.
X-ray crystallography can provide very detailed atomic information, showing every atom in a protein or nucleic acid along with atomic details of ligands, inhibitors, ions, and other molecules that are incorporated into the crystal. However, the process of crystallization is difficult and can impose limitations on the types of proteins that may be studied by this method. For example, X-ray crystallography is an excellent method for determining the structures of rigid proteins that form nice, ordered crystals. Flexible proteins, on the other hand, are far more difficult to study by this method because crystallography relies on having many, many molecules aligned in exactly the same orientation, like a repeated pattern in wallpaper. Flexible portions of protein will often be invisible in crystallographic electron density maps, since their electron density will be smeared over a large space.
Biological molecule crystals are finicky: some form perfect, well-ordered crystals and others form only poor crystals. The accuracy of the atomic structure that is determined depends on the quality of these crystals. In perfect crystals, we have far more confidence that the atomic structure correctly reflects the structure of the protein. Two important measures of the accuracy of a crystallographic structure are its resolution, which measures the amount of detail that may be seen in the experimental data, and the R-value, which measures how well the atomic model is supported by the experimental data found in the structure factor file.
Alt Text: Experimental electron density from a structure of DNA, revealing the atomic model generated from the data, showcasing the precision of X-ray crystallography in mapping electron densities.
3.2. Nuclear Magnetic Resonance (NMR) Spectroscopy
NMR spectroscopy is a technique that exploits the magnetic properties of certain atomic nuclei to determine the physical and chemical properties of atoms or the molecules in which they are contained. It relies on the phenomenon of nuclear magnetic resonance and can provide detailed information about the structure, dynamics, reaction state, and chemical environment of molecules.
NMR spectroscopy may be used to determine the structure of proteins. The protein is purified, placed in a strong magnetic field, and then probed with radio waves. A distinctive set of observed resonances may be analyzed to give a list of atomic nuclei that are close to one another, and to characterize the local conformation of atoms that are bonded together. This list of restraints is then used to build a model of the protein that shows the location of each atom. The technique is currently limited to small or medium proteins, since large proteins present problems with overlapping peaks in the NMR spectra.
A major advantage of NMR spectroscopy is that it provides information on proteins in solution, as opposed to those locked in a crystal or bound to a microscope grid, and thus, NMR spectroscopy is the premier method for studying the atomic structures of flexible proteins. A typical NMR structure will include an ensemble of protein structures, all of which are consistent with the observed list of experimental restraints. The structures in this ensemble will be very similar to each other in regions with strong restraints, and very different in less constrained portions of the chain. Presumably, these areas with fewer restraints are the flexible parts of the molecule, and thus do not give a strong signal in the experiment.
In the PDB archive, you will typically find two types of coordinate entries for NMR structures. The first includes the full ensemble from the structural determination, with each structure designated as a separate model. The second type of entry is a minimized average structure. These files attempt to capture the average properties of the molecule based on the different observations in the ensemble. You can also find a list of restraints that were determined by the NMR experiment. These include things like hydrogen bonds and disulfide linkages, distances between hydrogen atoms that are close to one another, and restraints on the local conformation and stereochemistry of the chain.
Alt Text: Restraints used to determine the structure of a small monomeric hemoglobin, illustrating how NMR spectroscopy provides data on atomic proximity and conformation.
3.3. Cryo-Electron Microscopy (Cryo-EM)
Electron microscopy, frequently referred to as 3DEM, is also used to determine 3D structures of large macromolecular assemblies. A beam of electrons and a system of electron lenses is used to image the biomolecule directly. Several tricks are required to obtain a 3D structure from 2D projection images produced by transmission electron microscopes. The most commonly used technique today involves imaging of many thousands of different single particles preserved in a thin layer of non-crystalline ice (cryo-EM). Provided these views show the molecule in myriad different orientations, a computational approach akin to that used for computerized axial tomography or CAT scans in medicine will yield a 3D mass density map. With a sufficient number of single particles, the 3DEM maps can then be interpreted by fitting an atomic model of the macromolecule into the map, just as macromolecular crystallographers interpret their electron density maps. In a restricted number of cases, electron diffraction from 2D or 3D crystals or helical assemblies of biomolecules can be used determine 3D structures with an electron microscope using an approach very similar to that of X-ray crystallography. Finally, 3DEM techniques are gaining prominence in studying biological assemblies inside cryo-preserved cells and tissues using electron tomography. This method involves recording images at different tilt angles and averaging the images across multiple copies of the biological assembly in situ.
In terms of molecular and atomic detail, both single-particle 3DEM and electron diffraction methods are now yielding structures at resolution limits comparable to macromolecular crystallography (i.e., enabling visualization of amino acid sidechains, surface water molecules, and non-covalently bound ligands). Cryo-electron tomography provides structural information at slightly lower resolution (i.e., protein domains and secondary structural elements). In calendar 2016, PDB depositions of 3DEM structures exceeded those coming from NMR spectroscopy for the first time.
Recent dramatic advances in the power of 3DEM reflect the convergence of a number of technologies, including sample preparation/preservation in vitreous ice, improved electron optics, phase plates to enhance electron image contrast, direct electron detectors, improved data processing software, and faster computers. This fortuitous convergence parallels the acceleration of macromolecular crystallography that occurred in the 1990s, when crystal freezing, synchrotron radiation beamlines, image plate and CCD detectors, improved data processing software, and faster computers came together in an earlier perfect storm for structural biology.
In work focused on very large macromolecular assemblies, where lower resolution is the norm, 3DEM data are increasingly being combined with information from X-ray crystallography, NMR spectroscopy, mass spectrometry, chemical cross-linking, fluorescence resonance energy transfer, and various computational techniques to sort out the atomic details. This practice of fusing multiple experimental approaches is often referred to as Integrative or Hybrid Methods (I/HM). They have proven very useful for multimolecular structures such as complexes of ribosomes, tRNA and protein factors, and muscle actomyosin structures.
Alt Text: Cryo-EM map of beta-galactosidase built from over 90,000 images, illustrating the detailed atomic model that can be derived from this technique.
3.4. X-ray Free Electron Lasers (XFEL)
New technology, termed serial femtosecond crystallography, is revolutionizing the methods of X-ray crystallography. A free electron X-ray laser (XFEL) is used to create pulses of radiation that are extremely short (lasting only femtoseconds) and extremely bright. A stream of tiny crystals (nanometers to micrometers in size) is passed through the beam, and each X-ray pulse produces a diffraction pattern from a crystal, often burning it up in the process. A full data set is compiled from as many as tens of thousands of these individual diffraction patterns. The method is very powerful because it allows scientists to study molecular processes that occur over very short time scales, such as the absorption of light by biological chromophores.
Alt Text: Structures of photoactive yellow protein determined by serial femtosecond crystallography, capturing the isomerization of the chromophore after it absorbs light.
3.5. Integrative or Hybrid Modeling (IHM)
Researchers are interested in studying larger and more complex systems, and use every technique available to do so. The structural biology community has had particular success in recent years by using an approach, termed “integrative modeling.” The idea is to combine information from a variety of methods, each good for studying a particular aspect of the system, to create an overall picture of the assembly.
For instance, combining spectroscopic or chemical crosslinking data that identify distances between components in an assembly, with low resolution electron microscopy data that give information on the overall shape of a complex, has become an effective strategy in integrative modeling. In addition to traditional structural biology methods such as X-ray crystallography, NMR spectroscopy and electron microscopy, experimental methods such as small angle solution scattering, Forster resonance energy transfer, chemical crosslinking, mass spectrometry, electron paramagnetic resonance spectroscopy, and other biophysical techniques have been used in integrative modeling studies. A key aspect of integrative modeling is that the resulting structural models do not always comprise of atomic coordinates and can contain regions of coarse-grained beads that represent multiple atoms. This is due to the fact that different kinds of experiments provide information at different levels of resolution.
An example of integrative modeling is the structure of the nuclear pore complex (NPC) from budding yeast determined using data from chemical crosslinking, small angle solution scattering and electron microscopy experiments. The NPC is an eight-fold symmetric assembly consisting of 552 copies of 32 different proteins belonging to the nucleoporin family. The overall shape of the NPC is obtained from a low resolution electron microscopy map. Extensive data from chemical crosslinking experiments provide information regarding the proximities and orientations of the nucleoporins within the assembly. Small angle scattering profiles for some of the nucleoporins are available and structures of several component nucleoporins and their sub-complexes have been obtained using experimental methods and/or computational modeling. All available information are gathered and combined together using computational algorithms to build the integrative model of the entire complex. This model of the NPC is archived in the PDB-IHM system for archiving structural models obtained using integrative or hybrid modeling and distributed by the wwPDB.
Alt Text: Cryo electron microscopy map of the Nuclear Pore Complex (NPC).
Alt Text: The integrative model of the Nuclear Pore Complex (NPC).
Alt Text: PDB structure of Nucleoporin.
Alt Text: PDB structure of Nucleoporin.
Alt Text: PDB structure of Nucleoporin.
Alt Text: PDB structure of Nucleoporin.
4. How Atomic Structures Influence Material Properties
The arrangement of atoms in a material dictates its physical and chemical properties. Understanding these relationships allows us to design materials with specific characteristics.
4.1. Mechanical Properties: Strength, Ductility, and Elasticity
The strength of a material is related to the forces between its atoms. Materials with strong interatomic forces, such as covalent or ionic bonds, tend to be stronger than materials with weak forces, such as van der Waals forces.
- Strength: The ability of a material to withstand stress without breaking.
- Ductility: The ability of a material to be deformed without breaking.
- Elasticity: The ability of a material to return to its original shape after being deformed.
4.2. Electrical Properties: Conductivity and Resistivity
The electrical conductivity of a material depends on the availability of electrons to move through the material. Metals have high conductivity because they have many free electrons.
- Conductivity: The ability of a material to conduct electricity.
- Resistivity: The resistance of a material to the flow of electricity.
4.3. Thermal Properties: Heat Capacity and Thermal Expansion
The thermal properties of a material are related to how it responds to changes in temperature. Heat capacity is the amount of heat required to raise the temperature of a material by a certain amount. Thermal expansion is the tendency of a material to change in volume in response to changes in temperature.
- Heat Capacity: The amount of heat required to raise the temperature of a material by a certain amount.
- Thermal Expansion: The tendency of a material to change in volume in response to changes in temperature.
5. Atomic Structures in Biology and Biochemistry
Atomic structures play a crucial role in biology and biochemistry, influencing the behavior and function of biological molecules.
5.1. Protein Structure and Function
Proteins are complex molecules made of amino acids linked together. The sequence of amino acids determines the protein’s three-dimensional structure, which is essential for its function.
- Primary Structure: The sequence of amino acids in a protein.
- Secondary Structure: Local structures such as alpha helices and beta sheets.
- Tertiary Structure: The overall three-dimensional structure of a protein.
- Quaternary Structure: The arrangement of multiple protein subunits in a complex.
5.2. DNA Structure and Function
DNA is the molecule that carries genetic information. Its double helix structure, with specific base pairing, is essential for its role in storing and transmitting genetic information.
- Base Pairing: Adenine pairs with thymine (A-T), and guanine pairs with cytosine (G-C).
- Double Helix: The two strands of DNA are twisted around each other to form a double helix.
5.3. Enzyme Mechanisms and Catalysis
Enzymes are biological catalysts that speed up biochemical reactions. Understanding the atomic structure of enzymes reveals how they bind to substrates and catalyze reactions.
- Active Site: The region of an enzyme where the substrate binds and the reaction occurs.
- Catalytic Mechanism: The step-by-step process by which an enzyme catalyzes a reaction.
6. Advances in Understanding Atomic Structures
Our understanding of atomic structures has advanced dramatically in recent years, thanks to new technologies and techniques.
6.1. Computational Modeling and Simulations
Computational modeling and simulations are increasingly used to study atomic structures and predict their behavior.
- Molecular Dynamics: A simulation technique that calculates the movement of atoms and molecules over time.
- Density Functional Theory: A quantum mechanical method used to calculate the electronic structure of atoms and molecules.
6.2. Advanced Microscopy Techniques
Advanced microscopy techniques, such as atomic force microscopy (AFM) and scanning tunneling microscopy (STM), allow scientists to image atoms and molecules at the atomic level.
- Atomic Force Microscopy (AFM): A technique that uses a sharp tip to scan the surface of a material and create an image of its atomic structure.
- Scanning Tunneling Microscopy (STM): A technique that uses a sharp tip to scan the surface of a conductive material and create an image of its atomic structure.
6.3. Synchrotron Radiation and Free Electron Lasers
Synchrotron radiation and free electron lasers provide intense beams of X-rays that can be used to study atomic structures with unprecedented detail.
- Synchrotron Radiation: Electromagnetic radiation emitted when charged particles are accelerated in a circular path.
- Free Electron Lasers: Lasers that produce intense pulses of coherent light by accelerating electrons through a magnetic field.
7. Future Directions in Atomic Structure Research
The field of atomic structure research is constantly evolving, with new discoveries and technologies emerging all the time.
7.1. Exploring Complex Materials and Systems
Future research will focus on understanding the atomic structures of more complex materials and systems, such as:
- Nanomaterials: Materials with structures on the nanometer scale.
- Biomaterials: Materials used in medical applications.
- Energy Materials: Materials used in energy storage and conversion.
7.2. Developing New Methods for Structure Determination
New methods for structure determination are constantly being developed, including:
- Improved Cryo-EM Techniques: Advances in cryo-EM technology are allowing scientists to study smaller and more complex structures.
- Combining Multiple Techniques: Integrating data from multiple techniques, such as X-ray crystallography, NMR spectroscopy, and cryo-EM, can provide a more complete picture of atomic structures.
7.3. Applying Atomic Structure Knowledge to Solve Real-World Problems
The knowledge gained from atomic structure research will be applied to solve real-world problems, such as:
- Developing New Drugs and Therapies: Understanding the atomic structures of disease-related molecules can lead to the development of new drugs and therapies.
- Creating Sustainable Materials: Designing materials with specific properties can help create more sustainable products and processes.
- Improving Energy Efficiency: Understanding the atomic structures of energy materials can lead to more efficient energy storage and conversion technologies.
8. Case Studies: Atomic Structures in Action
Let’s delve into specific examples where understanding atomic structures has led to significant breakthroughs.
8.1. The Development of High-Strength Alloys
By understanding the atomic arrangement in metals, materials scientists have developed high-strength alloys used in aerospace and automotive industries. For example, the addition of specific elements to aluminum can disrupt the regular crystal lattice, making it harder for dislocations (defects) to move, thus increasing the material’s strength.
Table: Composition and Properties of High-Strength Aluminum Alloys
Alloy | Composition | Tensile Strength (MPa) | Yield Strength (MPa) | Application |
---|---|---|---|---|
7075 | Al, Zn, Mg, Cu | 572 | 503 | Aircraft structures |
2024 | Al, Cu, Mg, Mn | 483 | 345 | Aircraft skin |
6061 | Al, Mg, Si | 310 | 276 | Automotive components |
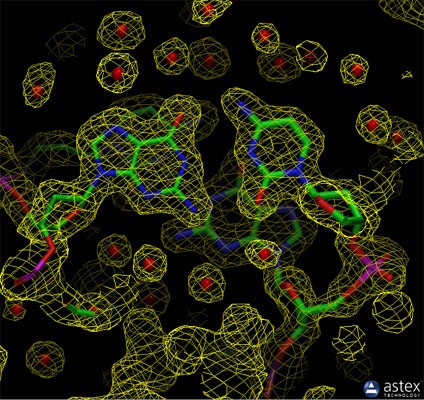
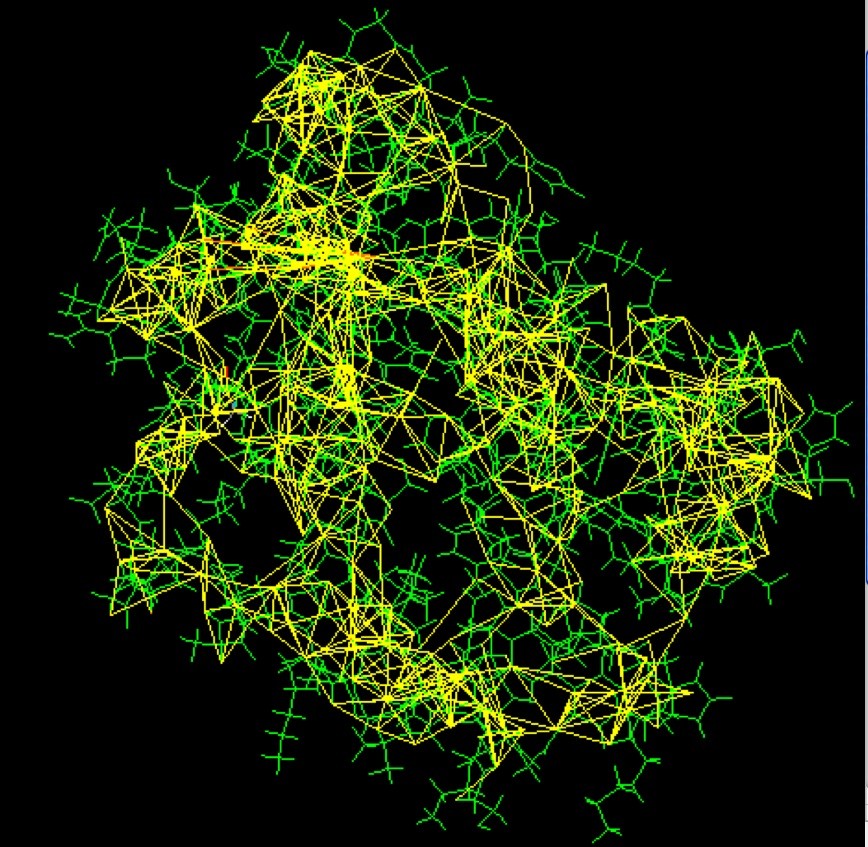
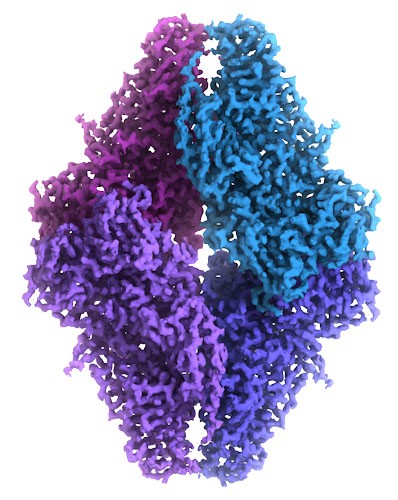
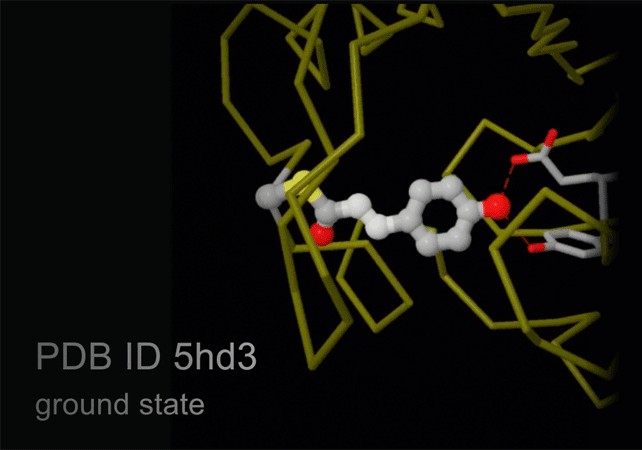
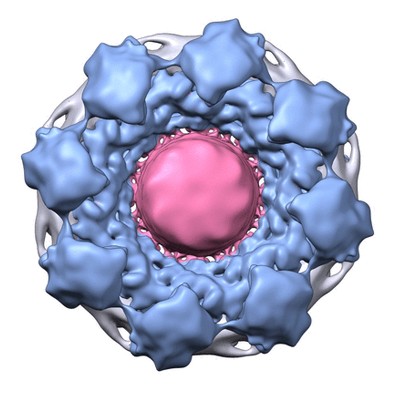
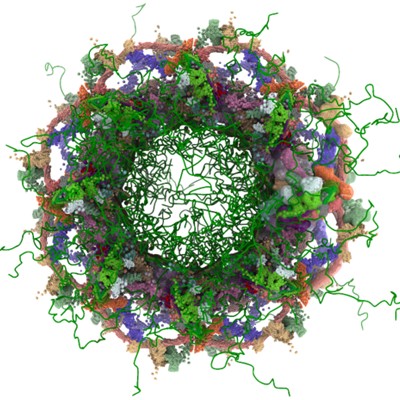
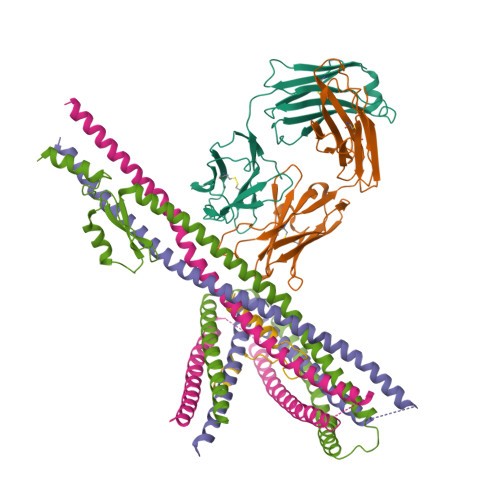
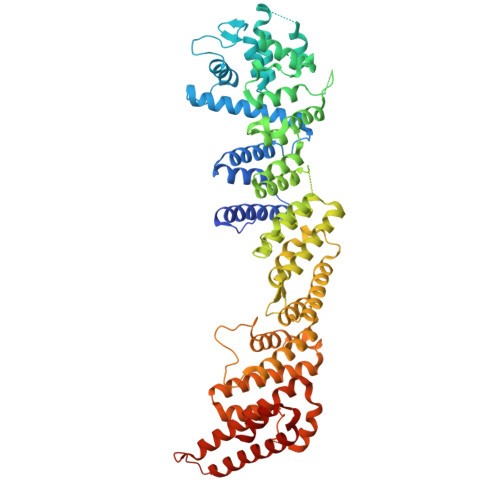
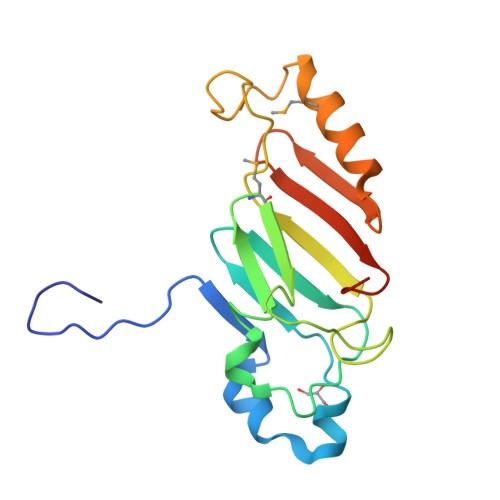
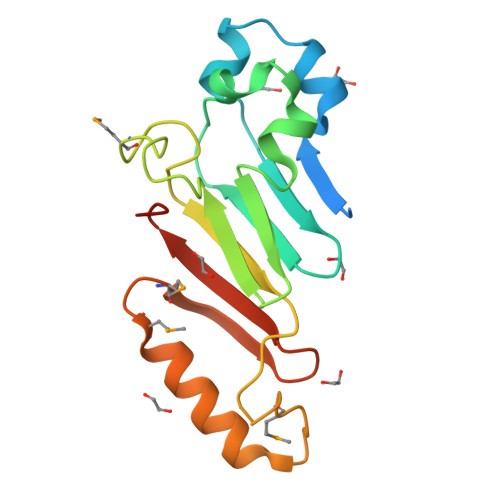
8.2. Understanding Enzyme Inhibition for Drug Design
Many drugs work by inhibiting the activity of specific enzymes. By determining the atomic structure of an enzyme and how a drug binds to it, scientists can design more effective drugs. For example, the structure of HIV protease was determined, leading to the development of protease inhibitors that are now a key part of HIV treatment.
8.3. Developing High-Efficiency Solar Cells
The efficiency of solar cells depends on the atomic structure of the semiconductor materials used. By understanding how different atomic arrangements affect electron flow, scientists have developed more efficient solar cells. For example, perovskite solar cells have shown great promise due to their unique atomic structure that allows for efficient light absorption and electron transport.
9. Tools and Resources for Learning About Atomic Structures
Several tools and resources are available to help you learn more about atomic structures.
9.1. Online Databases and Visualization Tools
- Protein Data Bank (PDB): A database of experimentally determined structures of proteins, nucleic acids, and other biological macromolecules.
- CCDC (Cambridge Crystallographic Data Centre): A database of small-molecule crystal structures.
- VMD (Visual Molecular Dynamics): A software package for visualizing and analyzing molecular structures.
- PyMOL: A molecular visualization program used to create high-quality images and animations of molecular structures.
9.2. Educational Websites and Courses
- LEARNS.EDU.VN: Offers comprehensive courses and resources on chemistry, materials science, and biology, covering atomic structures and their applications.
- Khan Academy: Provides free educational videos and exercises on chemistry and physics.
- Coursera and edX: Offer online courses on materials science, chemistry, and biology from top universities.
9.3. Textbooks and Scientific Literature
- “Inorganic Chemistry” by Shriver and Atkins: A comprehensive textbook on inorganic chemistry.
- “Physical Chemistry” by Atkins: A textbook on physical chemistry covering thermodynamics, kinetics, and quantum mechanics.
- “Biochemistry” by Lehninger: A textbook on biochemistry covering the structure and function of biological molecules.
- Journals like “Nature,” “Science,” and “Cell”: Publish cutting-edge research on atomic structures and their applications.
10. Career Paths Related to Atomic Structures
A strong understanding of atomic structures can lead to a variety of exciting career paths.
10.1. Materials Scientist
Materials scientists design and develop new materials with specific properties. They use their knowledge of atomic structures to create materials for a wide range of applications, from aerospace to medicine. The U.S. Bureau of Labor Statistics projects 5% growth in employment of materials scientists and engineers from 2022 to 2032.
10.2. Chemist
Chemists study the composition, structure, properties, and reactions of matter. They use their understanding of atomic structures to develop new chemical compounds and processes. According to the U.S. Bureau of Labor Statistics, the median annual wage for chemists and materials scientists was $87,450 in May 2023.
10.3. Biochemist
Biochemists study the chemical processes and substances that occur in living organisms. They use their understanding of atomic structures to understand the function of biological molecules and develop new drugs and therapies. The U.S. Bureau of Labor Statistics projects 4% growth in employment of biochemists and biophysicists from 2022 to 2032.
10.4. Structural Biologist
Structural biologists determine the three-dimensional structures of biological molecules, such as proteins and DNA. They use techniques like X-ray crystallography, NMR spectroscopy, and cryo-EM to visualize these structures at the atomic level.
10.5. Nanotechnologist
Nanotechnologists work with materials and devices at the nanometer scale. They use their understanding of atomic structures to create new technologies in fields such as medicine, electronics, and energy.
FAQ: Frequently Asked Questions About Atomic Structures
Q1: What is the basic structure of an atom?
An atom consists of a nucleus containing protons and neutrons, surrounded by electrons orbiting in specific energy levels or shells.
Q2: Why is understanding atomic structure important?
Understanding atomic structure is crucial for understanding the properties of matter, chemical reactions, and the function of biological molecules.
Q3: What are the main methods for determining atomic structures?
The main methods include X-ray crystallography, NMR spectroscopy, and cryo-electron microscopy (cryo-EM).
Q4: How does atomic structure affect material properties?
The arrangement of atoms in a material dictates its mechanical, electrical, and thermal properties.
Q5: What is the role of atomic structure in biology?
Atomic structures are essential for understanding the structure and function of proteins, DNA, and enzymes.
Q6: How are computational methods used in atomic structure research?
Computational methods are used to model and simulate atomic structures, predict their behavior, and analyze experimental data.
Q7: What are some career paths related to atomic structures?
Career paths include materials scientist, chemist, biochemist, structural biologist, and nanotechnologist.
Q8: Where can I find more information and resources on atomic structures?
Resources include online databases like the Protein Data Bank, educational websites like LEARNS.EDU.VN and Khan Academy, and textbooks on chemistry and biology.
Q9: How have advances in understanding atomic structure impacted drug design?
Understanding atomic structures of target molecules has enabled the design of more effective and specific drugs.
Q10: What are the future directions in atomic structure research?
Future research will focus on complex materials, new structure determination methods, and applying atomic structure knowledge to solve real-world problems.
Atomic structures are the foundation of all matter, and understanding them is essential for advancing science and technology. From designing new materials to developing life-saving drugs, the knowledge gained from studying atomic structures has a profound impact on our world. Join us at LEARNS.EDU.VN to delve deeper into this fascinating field and unlock the secrets of the universe.
Ready to expand your knowledge and explore the exciting world of atomic structures? Visit LEARNS.EDU.VN today and discover our comprehensive courses, expert instructors, and cutting-edge resources. Whether you’re a student, a professional, or simply curious, we have something for everyone.
Contact us:
Address: 123 Education Way, Learnville, CA 90210, United States
Whatsapp: +1 555-555-1212
Website: LEARNS.EDU.VN
Unlock your potential and embark on a journey of discovery with learns.edu.vn!